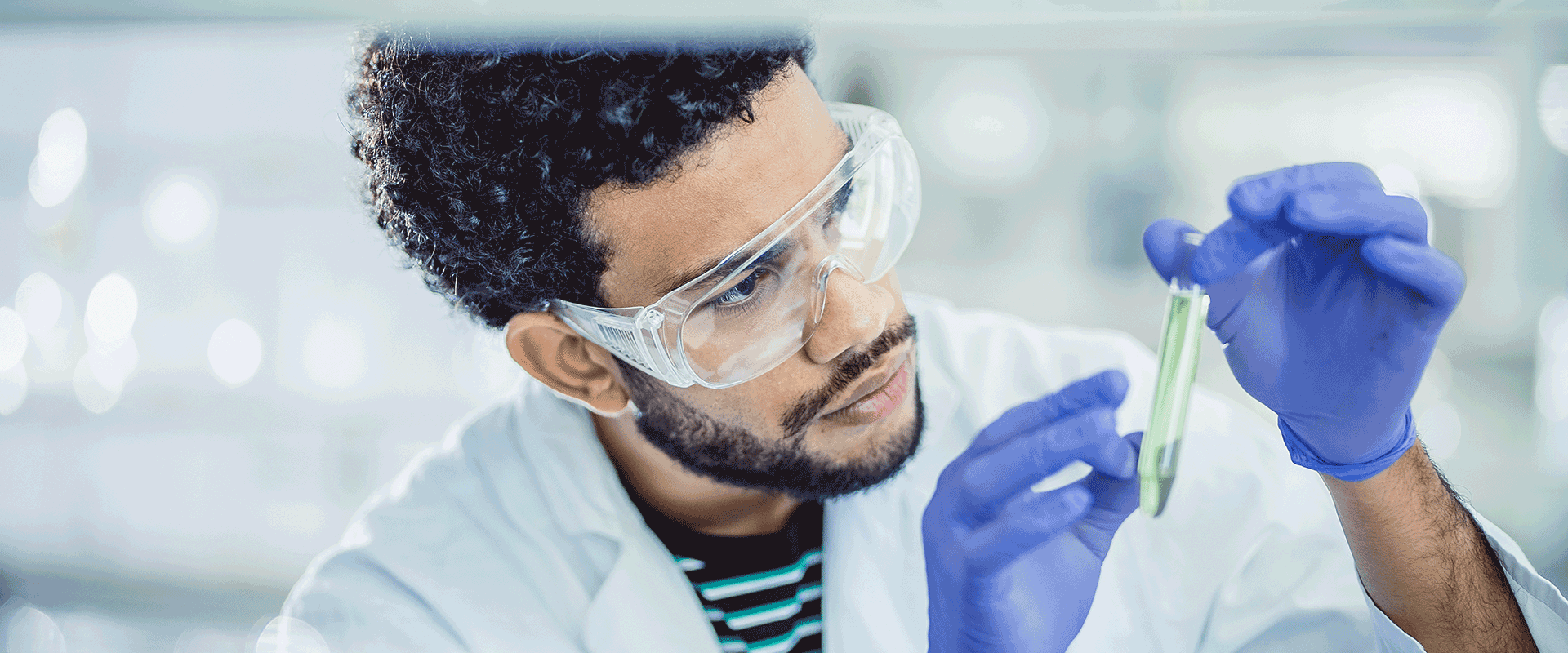
New Drug Discovery Paradigm: Advances in 3D Tissue Models and Applications
- Volume XXII, Issue 56
- Executive Insights
Just one in 10 drugs will make it from Phase I clinical trials all the way to FDA approval.
Advances in 3D tissue models have yielded new approaches, such as organ-on-a-chip systems.
Manufacturers of 3D tissue models are harnessing high-fidelity biology within specific disease model systems to support biopharma R&D in key therapeutic areas.
The promise of 3D tissue models extends beyond drug screening to novel drug target discovery and expedited drug design, as well as the identification of personalized medicine trial populations.
Both biopharma companies and enablers need to address a series of questions as 3D tissue models gain traction.
Despite ongoing technological advances, the current drug discovery paradigm is largely inefficient given that some 90% of investigational drugs fail in clinical development — and that’s in addition to the approximately 80% of drugs that fail in preclinical development before reaching Phase I trials.1 An estimated 40% of drug development costs today are associated with preclinical studies, and only a fraction of compounds advance to the clinic2, largely due to the lack of translatability of preclinical safety and efficacy data to humans.
To address this inefficiency, new advances in stem cell technology, microfluidics and tissue engineering have begun to yield tissue and organ models that are physiologically relevant (in other words, translatable) and scalable, setting the stage for a paradigm shift in drug discovery. These solutions should increase the probability of new medicines reaching patients while simultaneously reducing the cost and time of development and supporting animal welfare.
In this Executive Insights, we discuss recent advances in the field of 3D tissue models and their potential to improve the drug discovery paradigm.
Market context and technology trends
Drug discovery remains an inefficient process, as only one in 10 drugs will make it from Phase I clinical trials to Food and Drug Administration (FDA) approval. With this in mind, drug developers have continued to implement innovative new technologies to optimize their drug discovery and development efforts. Advances in such technologies as silico modeling, high content imaging and multiparametric analysis (e.g., impedance and kinetic-based measurements) have enabled more precise and nuanced discovery at greater biological depth. Despite these advances, however, the translatability of the data generated is limited due to a dearth of human-relevant physiology within the in vitro models that are used to test new medicines in early screening. In fact, most therapeutic areas still lack predictive and truly translatable biological models that can reliably generate early safety and efficacy insights that accurately inform the potential clinical performance of a drug.
As a mainstay of conventional drug discovery, in vitro monolayer cell-based assays (e.g., 2D) are used for large-scale screening and discrete interrogation of molecules. Most of these studies rely on immortalized cell lines that are both well characterized and abundant. Such homogenous cell populations can provide reproducible results in the context of high-throughput compound screening. However, these commonly used cell-based assays are often of limited utility due to their lack of translatability and their inability to predict how a drug will act at the tissue or system level when introduced into patients.
Efforts to move toward more translatable data within cell-based models have led to the use of primary human cells, which more closely resemble in vivo biology, thus improving clinical relevance. However, primary cells are obtained from patients/donors, a process that is often difficult and costly and generally lacks scalability due to sourcing challenges and heterogeneity issues within the samples.
In contrast to cell culture, animal models are inherently more complex and physiologically relevant, and are commonly used to support preclinical studies (e.g., ADME-Tox). However, species differences tend to hinder translation to human physiology and diseases. Small animal models, including genetically modified rodents, fail to capture the full spectrum of human disease pathology. Large animal models — which, depending on the disease, tend to better represent human biology (e.g., primates in central nervous system preclinical research) — present a variety of challenges, including high cost, long experiment timelines and various ethical considerations.
The limitations of current models illuminate the need for new tools and technologies that can combine the advantages of scalability and throughput seen in cell-based models with the ability to generate translatable data that incorporates the higher-level biology that animal studies can offer. With advances in stem cell research, induced pluripotent stem cells (iPSCs) can now be differentiated into cells of various lineages (e.g., cardiac, intestinal epithelial) with self-renewing properties. iPSCs enable maintenance of the phenotypic context of patients in a more scalable form, allowing drug discovery models that preserve the context of human diseases. These models can also be easily leveraged to explore patient subgroups and genetic variant effects with greater control and flexibility within the experimental system. Further, experiments based on iPSCs can be time- and cost-efficient while preserving animal welfare.
As an example of these novel models in use, Vertex Pharmaceuticals successfully utilized data from an in vitro cell-based model to gain FDA approval for expanding the indications for Kalydeco in cystic fibrosis. This was achieved by using engineered cells that harbor cystic fibrosis transmembrane conductance regulator (CFTR) genes and measuring the cellular response to Kalydeco in vitro. Researchers observed that cells engineered with more CFTR genes responded to Kalydeco and extrapolated the same clinical benefit observed in earlier clinical trials of other CFTR genes, but without the need to replicate the effect in patients.3
While the Vertex experience is relatively unique within the field of drug developers, it clearly illustrates the role that a well-validated model can play. Initial use cases are likely to manifest in ultrarare indications where patient recruitment could present challenges (e.g., in cystic fibrosis), but over time more advanced models are likely to offer broader advantages for efficient drug discovery and development efforts.
Recently, new platforms have emerged in the form of sophisticated 3D tissue models that can be trained to mimic the structure and function of human organs and better represent mature human biology and physiology when compared to previous 2D technologies. These models are capable of offering in vivo-like data at in vitro scale, which will enhance the research and discovery process by de-risking development through early efficacy/safety signals while simultaneously reducing costs and development time (see Figure 1). Going forward, we expect that these new technologies will continue to see greater validation and expanded use as researchers seek to leverage technologies in new ways, all the way up to the point of mirroring clinical trials in an in vitro model system.
Primary cells: Cells isolated directly from human tissues using enzymatic or mechanical methods. These cells have a finite life span and expansion capacity.
Immortalized cell lines: Differentiated cell populations that, due to mutations, proliferate indefinitely, allowing prolonged in vitro culture.
Small animal models: Small living animals used for research purposes. They require less space and are cheaper than large animals. Rodents (i.e., mice, rats) are used most commonly.
Large animal models: Large living animals used for research purposes. They are used primarily when large amounts of tissue must be collected or an anatomic area of interest is more like that in humans. Examples include farm animals and nonhuman primates.
iPSCs (induced pluripotent stem cells): Genetically modified adult cells that convert into pluripotent cells.
3D tissue models: Microenvironments where multiple cells (mostly iPSCs) are organized and stimulated to mimic in vitro, native tissue form and function.
A variety of approaches have been developed in the field of 3D tissue modeling. These include organ-on-a-chip systems that leverage electromechanical, microfluidic and other technologies to create an environment for cells and tissues (including iPSC-derived tissues) to mimic human organ functions.
Enablers in this field have emerged primarily from basic research and are harnessing high-fidelity biology within specific disease model systems to support biopharma R&D in key therapeutic areas (e.g., neurodegenerative, liver/toxicology, kidney, gastrointestinal, oncology, cardiovascular). While the majority of these companies, among them AxoSim and TARA Biosystems, focus on a specific model system, several — such as InSphero and Mimetas — have platform technologies that can support a variety of applications. We have featured a curated selection of the most validated solutions (see Table 1).
As these companies have evolved, their business models have also changed to meet customer needs. Companies with in-depth biology expertise have functioned as service providers for biopharma and can provide key R&D insights for a range of customers. Other companies have expanded beyond services to provide off-the-shelf kits with specialized consumables to support a broader set of customers and applications.
AxoSim has developed two novel neurology platforms: the Nerve-on-a-Chip, which mimics Schwann cell myelination and human-relevant electrical and structural metrics, and Mini-Brain, a 3D technology with human-relevant myelination. These solutions replicate the nuanced biology of the central and peripheral nervous systems, allowing researchers to support drug R&D across a number of neurodegenerative disease applications.
Emulate has developed a range of organ-specific solutions, including its Lung-Chip platform, which emulates the structure and biology of the lung. These specialized lung models offer researchers the ability to study biology of the alveolus (Alveolus Lung-Chip) and inflammation in the airway (Airway Lung-Chip) for diseases such as asthma and chronic obstructive pulmonary disease.
InSphero has engineered assay-ready 3D microtissue platforms, including proprietary processes, assay plates and an organ-on-a-chip technology for customized applications in liver toxicology, metabolic diseases and oncology. Among its solutions, InSphero develops human liver microtissues based on a coculture model of hepatocytes and Kupffer cells that support the prediction of drug-induced liver injury to provide improved compound classification, advance prioritization and de-risk development due to toxicity issues (a frequent problem for liver diseases).
Mimetas has engineered the OrganoPlate, a proprietary, microfluidic, 3D cell culture plate. It employs a liquid handling technology (Phaseguide) to enable barrier-free definition of culture matrices and cells in 3D, supporting cell-cell interactions and improved imaging and quantification. For example, OrganoPlate culture plates can be used to modulate the proximal tubule as a way to better understand its functionality and enhance the drug discovery process for renal ischemia and polycystic kidney disease.
Nortis has developed microfluidic chips designed to generate a variety of tissue architectures. The solution is based on a perfusion system in which platforms consisting of chips and supporting media reservoirs are integrated to create vasculature and simulate the human tissue microenvironment. Recent applications include the investigation of the mechanisms of an established nephrotoxin in human organs by testing it in a liver-on-a-chip and a kidney-on-a-chip, both developed by Nortis and linked to each other. The technology provides an ex vivo approach for investigating organ-organ interactions and drug toxicity characterization.
SynVivo has developed microfluidic chips to simulate microvascular networks. Its blood-brain barrier model recreates an in vivo microenvironment by emulating a histological slice of brain tissue in communication with endothelial cells across the blood-brain barrier. The model mimics the cellular makeup of desired cell populations to enable rapid drug screening, resulting in improved discovery and toxicity analysis as well as mechanistic insights into drug transport in these tissues.
TARA Biosystems has harnessed the advances of iPSCs and tissue engineering to develop a novel and scalable platform of mature heart muscle tissue for cardiovascular research. These engineered heart tissues mimic the cardiac physiological microenvironment by recapitulating the structure and function of adult heart muscle (e.g., electrical impulses driving calcium handling and therefore heartbeat), a key characteristic that is lacking in traditional cardiac cell lines. TARA’s platform can address cardiovascular drug discovery and development and potentially replace costly in vivo animal cardiac safety studies. TARA is also expanding its platform for skeletal muscle tissue models.
Three-dimensional tissue and organ models are seeing increased adoption and validation within the market, and key enablers are rapidly undertaking new R&D efforts to expand models’ usability and drive broad market use of their technologies. Efforts are underway to demonstrate equivalence to established models as a prerequisite to broadening adoption among drug developers as well as to drive acceptance by regulatory frameworks. Such advances will help validate these novel approaches and encourage their use among investigators. Further, the FDA has outlined initiatives for incorporating novel model systems, including the Center for Drug Evaluation and Research’s Model-Informed Drug Development Pilot Program, which aims to integrate diverse data sources to help decrease uncertainty and lower failure rates; the Predictive Toxicology Roadmap, designed to integrate predictive methods into safety/risk assessments; and the FDA-National Institutes of Health Biomarker Working Group, which has provided suggestions for the development of translational tools.4
The promise of 3D tissue models extends beyond drug screening. The utility of preclinical models is limited to their ability to recapitulate human physiology, but in many diseases the basic mechanisms driving pathology are unknown. Novel 3D tissue models allow multiscale evaluation of tissue form and function over a discrete time course, such as in the progression from healthy to pathological states. In doing so, these systems may better identify novel drug targets and expedite drug design while reducing costs and offering deeper translational insights that will inform drug effects in vivo.
This approach can be further applied in a precision medicine context to better delineate which patient subpopulations will respond to a therapy (e.g., to inform clinical trial design). As personalized medicine advances, preclinical models will need to evolve to represent more and more targeted patient populations. IPSC-derived tissue models might offer biopharma companies the ability to identify patient subpopulations that could potentially best respond in a clinical trial very early on in the drug development process, as these models would allow them to run a “clinical trial in a dish” using genetically defined or patient-derived cells in vitro before spending millions of dollars on a human study.
As 3D tissue models continue to gain traction, key questions will need to be addressed by both biopharma and enablers.
Questions for biopharma:
Questions for enablers:
Endnotes:
1BioMedTracker Clinical Development Success Rates 2006-2015; DiMasi et al., J Health Economics (2016).
2Paraxel Biopharmaceutical R&D Statistical Sourcebook 2018/2019.
3Ratner, M. “FDA deems in vitro data on mutations sufficient to expand cystic fibrosis drug label.” Nat Biotechnol 35, 606 (2017).
4Food and Drug Administration.